News
Published 15 November 2021World Antimicrobial Awareness Week: Professor David Ackerley
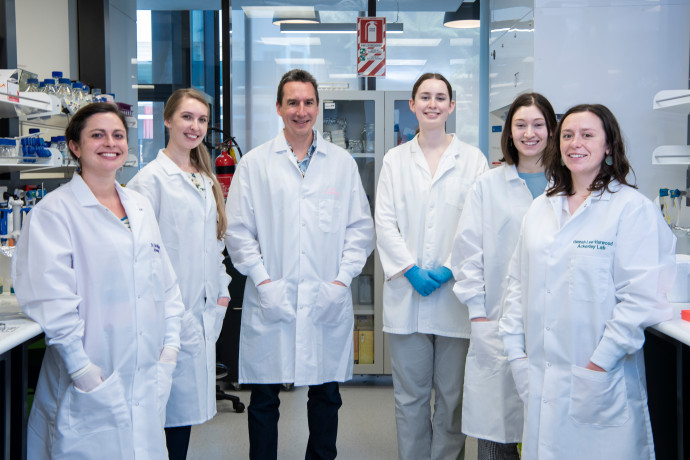
World Antimicrobial Awareness Week (WAAW) is celebrated annually to increase global understanding of antimicrobial resistance. This year we're highlighting researchers in Aotearoa working to raise awareness on antimicrobial resistance.
Professor David Ackerley is a microbiologist, enzyme engineer, and Biotechnology Programme Director at Te Herenga Waka—Victoria University of Wellington. His primary research focus is on the discovery, characterisation, engineering, and application of useful bacterial enzymes, and novel antibiotics to counter the spread of multi-drug resistant bacteria.
David is currently working alongside Dr Elise Williams, Dr Abby Sharrock, Hannah Lee-Harwood (PhD student), Jenni Francis (PhD student) and Alex Linton-deBoer (undergraduate summer student) from the Microbial Biotechnology Lab (at Victoria University) on the project 'Forewarned is forearmed: studying how antibiotic resistance can evolve', which is supported by Te Pūtea Rangahau a Marsden the Marsden Fund.
Q: What does your current research involve and how are you working to understand antimicrobial resistance?
A: Most people are aware that DNA is the information stored in cells that acts as the genetic blueprints for life. But what does that actually mean, and how does it allow life to evolve to meet new challenges?
The information encoded by individual genes in our DNA is used to build individual proteins - a vast number of them, which play diverse roles in cells. Many of the most essential proteins are enzymes – tiny nanomachines that perform virtually all of the chemistry of life, including replicating the DNA and helping to build other proteins. Often scientists who study this chemistry think about each enzyme as contributing a very specific function to our cells. However, evolutionary history is a noisy and tumultuous affair, and a function that is critically important for a cell today may have been virtually irrelevant millennia ago. It is becoming clear that enzymes that have some capacity to “moonlight”, i.e. perform additional jobs beyond their current primary role, can buffer cells against new stresses that arise. If the new stress persists, the enzyme can adapt, honing their new skills and turning the moonlighting job into a fixed full-time role.
This type of enzymatic career change can be particularly important for bacteria, which cannot run away from new stresses but must either evolve to meet the challenge or risk extinction. A classic example of this is the development of antibiotic resistance. In complex environments such as soil, which can harbour over 10,000 different bacterial species per gram, microbes collectively produce a bristling array of antibiotics as weapons. These enable them to battle with other species that are competing for the same limited resources. Bacteria that can withstand the onslaught of a new antibiotic have a competitive advantage in this life-or-death struggle, so a moonlighting enzyme that just happens to be able to detoxify a particular antibiotic may provide a critical edge.
In natural environments, bacteria are confronted with an ever-changing host of enemies that all make different antibiotics, so there is seldom the sustained pressure required to adapt an amateur moonlighting enzyme into an antibiotic resistance specialist. But humans have changed the game by repurposing nature-derived antibiotics to treat clinical infections. Suddenly, antibiotics are being produced and used on a scale the world has never seen – and if used inappropriately, e.g. by patients who fail to complete a course, then the bacteria containing the most effective moonlighting enzymes may survive and move one step closer to developing full immunity to that antibiotic.
With support from the Marsden Fund, our team is studying how entirely new forms of antibiotic resistance can develop from moonlighting enzymes. Studies like this are usually performed by choosing a single disease-causing bacterium and growing it over many weeks in the presence of incrementally-escalating doses of an antibiotic. However, this approach would rely on the target bacterium already containing a suitable moonlighting enzyme and does not allow for the fact that bacteria typically live in mixed communities that are extremely good at sharing genes. In the environment, it is more likely that resistance will arise in a different bacterium and then be passed to the disease-causing strain.
To recognise this, we are focusing on soil as a rich model environment and purifying all of the DNA from the thousands of bacterial species that reside there, en masse. This provides access to the collective set of genetically-encoded enzymes, and we have developed a unique way of breaking this DNA up into small gene-sized fragments and maximising the amount of protein they will produce when transferred into a tame laboratory bacterium called E. coli. Because we can get high-level production of the new enzymes – often comprising ~10% of the total E. coli protein output – we are able to detect very weak moonlighting activities that help defend their new E. coli home against an antibiotic challenge. We can then use artificial evolution techniques to evaluate whether these have the propensity to adapt into dangerous levels of antibiotic resistance. Of course, we have to be very careful in doing so, as we want to understand the spread of antibiotic resistance, not contribute to it! And so, all our work is conducted under the same physical containment conditions as used to study disease-causing bacteria that are already antibiotic resistant.
In addition to understanding the chemistry of antibiotic resistance, our work provides insight into how readily resistance to new antibiotic drugs might arise in the clinic, and the most likely way(s) for this to happen. This can in turn inform possible counter-measures. For example niclosamide, a drug currently used to eliminate tapeworms, has recently been recognised as a promising anti-bacterial and is in clinical trials to treat a variety of skin infections. Traditional dose-escalation studies using individual bacteria have suggested that spontaneous resistance is unlikely to arise, but our studies have identified several different types of enzyme with a moonlighting ability to detoxify niclosamide using a chemical reaction called “nitro-reduction”. Worryingly, we have shown that this moonlighting activity can easily be evolved to protect bacteria against the highest achievable doses of niclosamide that could be administered to patients. However, we have also shown that the effects of some other antibiotics including metronidazole – a very common drug you may well have taken yourself at some point – are strengthened by nitro-reduction. So, by co-administering metronidazole with niclosamide, doctors should be able to prevent this form of resistance arising.
David Ackerley
Professor
With support from the Marsden Fund, our team is studying how entirely new forms of antibiotic resistance can develop from moonlighting enzymes.